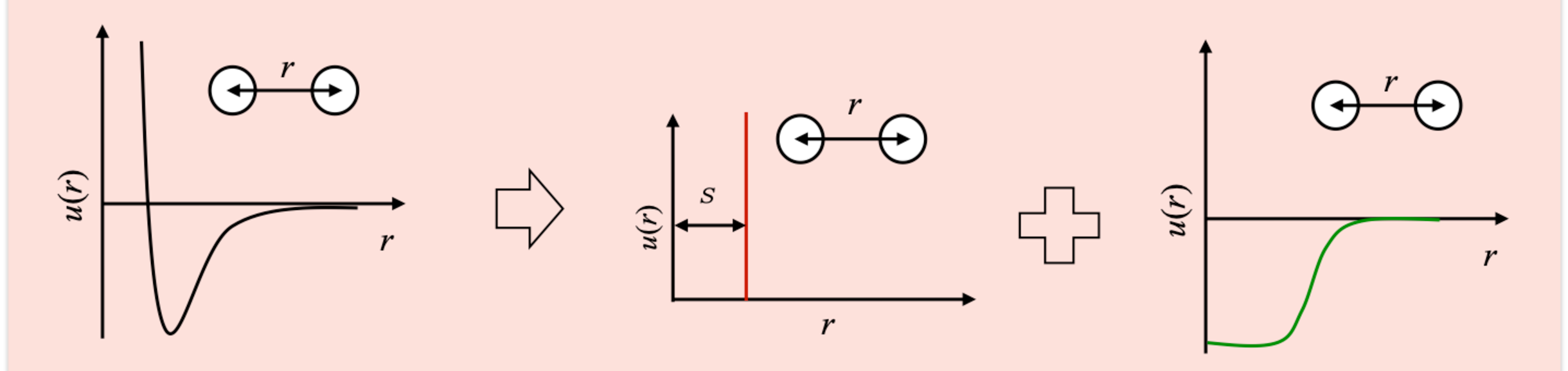
Research
Research Interests
-
Integrating Physics and Machine-Learning Models for Inverse Materials Design (NSF)
Recent years have seen tremendous efforts on rational materials design, However, progress has been limited due to not only the complexity of materials synthesis but the challenge to discover new materials that meet diverse technical and economic constraints pertinent to their practical use. From a computational perspective, brute-force molecular simulations and first-principles methods are often too expensive for large-scale search of the chemical space, while modern data-driving approaches are severely hampered by the scarcity of high-quality training data. We propose to solve these challenges by using recent advances in physics-based modeling and data science. The overarching goal is to establish efficient, systematic, yet accurate computational tools for inverse design of nanostructured materials useful for energy storage and chemical separation.
-
Theory and application of polyelectrolyte complexation (NSF)
Interaction of polyelectrolytes with oppositely charged substances results in various self-assembled structures commonplace in industrial settings and biology. While there is a large body of experimental work on polyelectrolyte complexes, much lagged behind is a theoretical description of the complex structure and thermophysical properties important for engineering applications or biological functions. The proposed research aims to develop predictive theories that can be used to describe polyelectrolyte complexation from a molecular perspective. The theoretical tools will be applied to examine the role of electrostatics and other non-specific intermolecular interactions in genome packaging and formation of viral capsids.
The techniques to be employed in this research is built upon recent work in the PI’s group in development and application of classical density functional theory (DFT), which provides a unifying computational tool for describing the microscopic structure and interfacial behavior of complex molecular systems. It has been demonstrated that the DFT captures the local packaging and long-range correlations due to electrostatic interactions and intra-chain structure that are essential for successful description of polyelectrolyte complexes. On the theoretical end, this project will address the effects of molecular topology and solvent-mediated non-electrostatic interactions on complexation of polyelectrolytes with oppositely charged surfaces, macromolecules or surfactants. The theoretical performance will be calibrated with Monte Carlo simulations for simplified models of polyelectrolyte complexes planned in this work and from the literature. In parallel with the theoretical developments, the applied work seeks a quantitative understanding of genome packaging and viral replication in hepatitis B virus. The particular viral system is selected not only for its obvious biomedical implications but also for its well quantified microscopic details already established by microbiologists that make it ideal for a stringent test of emerging molecular modeling techniques. Application of DFT to viral packaging will be in collaboration with experimental groups from the medical schools at the Pennsylvania State University and at the University of Texas.
Accomplishments from this research will further theoretical descriptions of the structure and thermodynamic properties of complicated polymeric systems from a molecular perspective. The generic techniques will be useful not only for describing the properties of polyelectrolyte complexes per se; they will also have impacts in the broader fields of complex fluids and molecular engineering. In particular, the computational tools developed in this work may have unusual impacts in fundamental research toward understanding the molecular basis of myriad biological processes that entail polyelectrolyte complexation of DNA/RNA chains or unstructured protein domains. Such understanding is essential, for examples, in developing of effective drugs for treatment of virus-induced contagious diseases and in formulation of efficient gene/biopharmaceuticals delivery systems.
-
Quantification of genome packaging in HBV nucleocapsids (NIH)
Hepatitis B virus (HBV) infects more than 2 billion people alive today and is responsible for over 1 million deaths caused by acute and chronic hepatitis and hepatocellular carcinoma every year. Although remarkable progress has been made in understanding the natural history and pathogenesis of HBV infection, effective treatment and eradication of chronic HBV infection remain a tremendous therapeutic challenge. The next biomedical breakthrough towards a cure is awaiting innovative interdisciplinary approaches to examine the detailed mechanism responsible for HBV infection and replication from a molecular perspective. The planned research introduces novel molecular modeling methods combined with in vitro X-ray/neutron scattering experiments to quantify the internal structure of HBV nucleocapsids and electrostatic regulation at various stages of the viral replication. Specific aims include: 1) To determine the density distributions of the genomic nucleic acids and the C-terminal domain (CTD) of the core protein and their potential changes during NC maturation; 2) To elucidate the effect of electrostatic interactions and the CTD phosphorylation state on NC maturation and stability. Theory and experiment will create a synergy because, on the one hand, the scattering experiments provide necessary data for calibration of theoretical predictions and, on the other hand, computational results will further understanding the genome packaging in greater details. Theoretical calculations will also provide information not directly accessible by scattering experiments such as structural and thermodynamic properties pertaining to capsid formation and stability in vivo. By investigating biomolecular interactions within HBV nucleocapsids that underpin the viral replication, accomplishments from this work will help to develop unconventional system-based therapeutics to regulate and ultimately eradicate HBV replication. If successful, the generic nature of the proposed computational and experimental methods ensures that this research will have unusually high impact in understanding the molecular basis of viral morphogenesis and in developing drugs for effective treatment of virus-induced contagious diseases.
-
Condensation and icing at superhydrophobic surfaces (NSF)
Superhydrophobic surfaces are promising for a wide variety of interesting applications owing to their extremely high water contact angles (typically greater than 150°) and low hysteresis (<5°). Up to date, most published researches on superhydrophobicity are focused on the effects of surface topology on water contact angle by trapping macroscopic air pockets at geometrically heterogeneous surfaces. While the extraordinary water repellency of a superhydrophobic surface can be successfully described by various modifications of Young’s equations, the macroscopic approach is insufficient to describe surface phase transitions affiliated with broader applications of superhydrophobic surfaces including anti-fogging and/or anti-icing. Such broader applications hinge on a better understanding of the surface hydrophobicity that depends on the microscopic details of the substrate and different states of water at inhomogeneous conditions. By bringing together complementary expertise, the proposed collaborative research aims to (i) establish a theoretical framework for describing vapor-liquid condensation, wetting transition and heterogeneous ice formation at superhydrophobic surfaces, (ii) develop practical schemes for fabrication of superhydrophobic surfaces with systematically tailored surface morphologies and chemical properties, (iii) conduct real-time study of condensation on superhydrophobic surfaces by a high-resolution environmental scanning electron microscope with atomic force microscope and cooling stage attachments, and (iv) study icing of supercooled water on superhydrophobic surfaces using a custom-designed experimental system.
-
Computational design of novel electrical energy storage systems (DOE)
The rational design of novel electrical energy storage (EES) systems with high energy and power density will require fundamental research and methodological developments. Whereas last few decades have seen tremendous progress in modeling the equilibrium and microscopic structures of EES systems at idealized conditions, predictive models for charge transport and redox reactions under nanoconfinements are largely undeveloped. The underlying causes of the impressive capacitance enhancement observed for pores smaller than 1 nm is not completely understood nor are the limits of how far this phenomenon can be extended. The overarching objective of our computational modeling is to establish a full suite of computational techniques to predict not only the static properties of electroactive materials but also charge transport and stability of these materials during charge-discharge cycling.
Many opportunities exist to develop and apply new continuum, statistical-mechanical, atomistic, and quantum-mechanical models. Although this effort can benefit from progress in related fields, rational design of EES systems presents many unique modeling challenges, such as ultra-fast, nonlinear, and nonuniform double layer charging; anomalous ion transport and wetting characteristics in nanopores; electrode materials with poor electron conductivity, pseudocapacitance combining redox reactions with charge screening; and widely disparate length and time scales from molecular events to device performance. To achieve the ideal of rational design of EES materials, microstructures, and systems, we will develop fundamentally new theories and modeling tools that allow us to describe nonlinear dynamics of electric double layer charging beyond the Poisson-Nernst-Planck equations and charge storage via multiple mechanisms, for example, through reversible redox reactions, adsorption of conformal dielectric or conducting materials, or incorporation of a dielectric material onto high conducting substrates. Conductive coatings of materials with pseudocapacitive behavior, such as conducting polymers, will dramatically increase the electrode’s capacitance. Poor electron conductors may be of particular interest as electrode materials because they are often strongly bonded, giving them good stability and safety characteristics. Methods to model mixed conduction in these systems need to go beyond mean field theory to explain the effect of the strong coupling between localized polarons and ions on the overall transport characteristics. Such transport models will need to be informed by electronic structure methods beyond common electronic to properly capture electron localization.
In this project, will address central questions related to the optimum performance of EES systems by considering two types of model systems. We will describe transport and structural properties of ionic systems within and along the electrochemical double layer using both theoretical modeling and data-based methods. In collaboration with experimental and theoretical groups, we will use the classical density functional theory (cDFT) to unravel various effects on ion and solvent transport due to application of a surface electrical potential or gate potential. For example, cDFT allow us to obtain chemically equilibrated water/electrolyte/interface structures in the presence and absence of external electric fields. Molecular-scale insights into the driving forces behind the material response to the applied electric potential will be obtained from a combination of physics- and data-based simulations.
-
Modeling the interfacial behavior of oil/brine/rock systems (PRF)
This project seeks to understand, and ultimately control, the interfacial properties of oil/brine/rock (COBR) systems and their influences on the wettability of reservoir rocks. To attain a quantitative description of the phase and interfacial behavior of reservoir fluids, we will explicitly consider various molecular driving forces underlying the interfacial behavior including the effects of multivalent ions on electrostatic interactions, surface chemistry, and the crude oil and brine compositions. Through validation with experimental observations and systematic investigation of all possible mechanisms of wettability reversal, our overarching goal is to provide an integrated approach to describe various physicochemical interactions taking place at oil/brine, brine/rock, and oil/rock surfaces essential for understanding, and eventually predicting, wettability changes in response to salinity water flooding (LSW) recovery.